Yamaha Motor Company
Yamaha Motor Company wanted to improve performance of its off-road motorcycle radiator assemblies and was looking for a way to reduce time-consuming real-world testing.
Simulation for Yamaha is an effective means of identifying locations for strength countermeasures and for developing new and more-effective designs
If an off-road motorcycle topples over at low speed during a competition, minor damage can accumulate that affects the motorcycle’s performance. For example, the impact from the motorcycle hitting the ground can deform the radiator assembly enough to reduce cooling performance, cause a coolant leak - or both.
Installing a plastic side cover helps protect the radiator assembly, and both the radiator and the plastic cover can withstand deformation if the motorcycle falls on its side at low speed. But Yamaha Motor Co., Ltd.’s engineers found that identifying exactly where to strengthen the radiators and plastic covers in its liquid-cooled, off-road motorcycles was requiring a high degree of trial and error.
“We would have to perform a lot of physical testing to identify the locations for countermeasures, including plastic cover design and thickness, radiator bracket, and bolt locations,” explains Mr. Masakazu Yamaya, Supervisor, Research & Development Section, Technology Center, Yamaha Motor Co. “This testing to analyze the strength of real-world vehicles would consume a tremendous amount of time and prototype parts. It would also make physical testing expensive and prone to human error.”
So Yamaha decided on a different, simulation-based approach, and carefully verified their new analysis methodologies through simplified laboratory testing. “After building various physical test setups to establish baselines for what we needed to know for damage assessment, we developed computer simulations of the physical tests,” explains Mr Yamaya. “We realized that if our simulations correlated well with the results of the physical tests, then the simulations would be an effective design-support tool.”
In a Yamaha liquid-cooled off-road motorcycle, the cover protecting the radiator assembly typically hangs off the side of the motorcycle frame. The radiator is a brazed thin-walled aluminum part protected by a plastic side cover. This arrangement provides enough strength for the radiator to withstand damage when the cover strikes the ground at very low speeds; namely, those inevitable - and often embarrassing - times when a motorcycle tips over. Unfortunately, a motorcycle toppling onto a hard surface is rarely righted unscathed; radiator fins and core supports are often bent or broken, and the protective plastic cover is often cracked or shattered to pieces.
“We needed to determine what was important to include in our toppling simulations, including parts, forces, and movements,” says Mr Yamaya. “We then had to determine what forces related to what results. Finally we had to confirm the accuracy of the simulations against the results of physical tests to determine the strength of the radiator assembly and the plastic cover - individually and mounted together - at various loadings, in order to accurately simulate impacts and impact speeds.”
Using a push jig, Yamaha’s first bench test focused on reproducing the damage to the radiator and plastic cover, and identifying the locations where strength countermeasures were necessary. The test measured the reaction force of the radiator assembly as a proxy for the strength of the radiator and plastic cover. The test was performed with two models of radiator assembly that differed in the design and the thickness of the side cover.
The bench test successfully reproduced the damage to the radiator and the buckling of the plastic cover in a motorcycle that has toppled over. The test also identified dents in the radiator components that were thought to have been caused by surrounding parts. To duplicate the damage situation on the radiator and plastic cover after a tip-over, Yamaha added the bottom radiator part that touches the ground to the assembly.
Another bench test analyzed the strain rate sensitivity in the plastic cover. Tests were run at three pushing speeds between 10 mm/min. and 500 mm/min. The results showed that the maximum load—the buckling load—of the plastic cover is also a function of speed. Moreover, the cover began to buckle when pushed by approximately 20% of the specified stroke in the push jig—regardless of the model of the radiator assembly.
“This meant two things to us,” says Mr Yamaya. “First, strain rate sensitivity needed to be in the simulations of the plastic cover. Second, the differences in the maximum reaction force in the different radiator models were essentially identical to those when the plastic cover began to buckle. Reproducing the buckling of the plastic cover would indeed accurately determine the maximum reaction force of the radiator assembly.”
For the radiator, the engineers ran two strength tests to determine the necessity of modeling the heat-radiating fins on that structure. One test applied to an ordinary radiator; the other, a radiator without fins. The tests showed the fins accounted for approximately 50% of the reaction force generated by the radiator. Although the thickness of each fin is only about 0.1 mm, the large number of fins together account for the greater effect on the radiator reaction force. Based on these results, Yamaha decided to include the fins in its strength simulation.
“All of these physical tests confirmed what components we needed to model in the digitized strength simulations,” explains Mr Yamaya. “The tests also provided baseline results for comparing the simulation model against the physical models.”
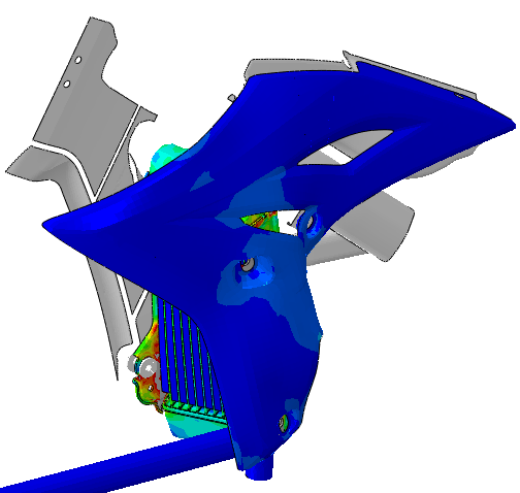
Developing Strength Simulations of Components
As Yamaha began creating their virtual models, they decided to run their simulations as quasi-static problems involving the dynamic explicit analysis methods found in Abaqus FEA from Dassault Systèmes’ 3DEXPERIENCE technology, SIMULIA. The explicit method was chosen because it is less computationally intensive than full implicit-type analyses when dynamically analyzing static problems. First the team developed separate cover and radiator models; then they combined them into full assembly models.
For the plastic cover, the simulation model was created using shell elements, the fuel tank was expressed as a rigid body, and the fuel tank reference node was fixed. Locations fastened by bolts were expressed by multipoint constraints, and constraints around the bolt center axis were free. The plastic cover and the fuel tank could interact with each other by contact definition. Multiple mounting points were constrained in the FEA model to forcibly vary the position of the reference node. Strain-rate sensitivity for the plastic material in the radiator cover was included using the test data for three different strain rates including the assumption of perfect plasticity at the highest strain rate.
The maximum load in the simulations was approximately 10% higher than that from the physical tests. “We determined this difference was because of the higher rigidity in the model of the plastic part where the thickness of the part’s geometry changes gradually,” says Mr Yamaya. However, the buckling seen in the plastic cover simulations matched well with the physical tests.
Shell elements were used to model all the radiator parts except its heat-radiating fins. The fins were expressed using multipoint constraints because creating the actual shapes of the fins and fitting them into the model of the radiator would have taken too much time and added unnecessary complexity to the simulation.
“We created the fins in Abaqus as a user material for honeycombs, based on real-world calibration of a fin unit model,” explains Mr Yamaya. “The simulation used a dynamic stress-strain relation that had the equivalent mechanical behavior as the fins. As a result, we were able to easily create the simulated fins with solid elements, and they more than adequately reproduced the response of the actual fin unit.”
In the simulations, the generated load was approximately 20% higher in the radiator model than in the physical push jig test. Mr Yamaya believes that the rigidity of the simulated radiator fins is higher than the actual fins because the model omits the slits machined onto the surface of the actual fins.
Verifying the Radiator Assembly Simulations
Finally, an integrated radiator assembly model was created to reproduce the correct interaction behavior between components, including the radiator and plastic cover (Figure 2).
Yamaha could then determine the total amount of internal energy and kinetic energy for the radiator, plastic cover, and full assembly of both components. “The percentage of total kinetic energy relative to total internal energy is on the order of several percent,” says Mr Yamaya. “This shows that a valid solution can be provided by addressing radiator assembly deformation as a quasi-static problem.”
In fact, he continues, “for the radiator, the same damage conditions of overall twisting were reproduced with nearly exact correlations between the physical test and simulation results. The buckled part of the plastic cover correlated perfectly as well, so we were confident to try out different designs for the radiator’s plastic cover.”
Based on these findings, Yamaha is now confident that its radiator assembly strength simulations can accurately predict physical test results. Using simulation, says Mr Yamaya, “provides us with a detailed analysis of the behavior of the radiator and plastic cover during physical test—behaviors that would otherwise be difficult to observe from testing alone. Simulation for Yamaha is an effective means of identifying locations for strength countermeasures and for developing new and more-effective designs”.